Olfaction: Minority odors get equal say
How well could you see a small green leaf if your world was enveloped in a bright purple haze? You would probably struggle to see it at all. But what if instead of detecting colors, you were detecting smells? In this case, you might detect the equivalent of the leaf pretty clearly, but hardly even notice the equivalent of the purple haze. Now, in eLife, Alexander Fleischman and colleagues – including Benjamin Roland, Rebecca Jordan and Dara Sosulski as joint first authors – report on the neural mechanisms that allow information about ‘minority’ odors to be detected through the overwhelming haze of another smell (Roland et al., 2016).
Scents are detected when odor molecules bind to odorant receptors on the surface of olfactory receptor neurons. These neurons then send signals to other neurons in a structure called the olfactory bulb, which processes this information and sends it to other olfactory regions in the brain.
To investigate the neural activity that underlies odor detection, Roland et al. – who are based at a number of institutions in France, Germany, the UK and the US – have revisited the ‘monoclonal nose’ mouse, an intriguing mouse model that was first reported in 2008 (Fleischmann et al., 2008). Mice normally express about 1100 subtypes of odorant receptor and scatter these evenly over several million receptor neurons (with each receptor neuron having one odorant receptor subtype). However, monoclonal nose mice express the same receptor (called M71) in 95% of their receptor neurons (Figure 1).
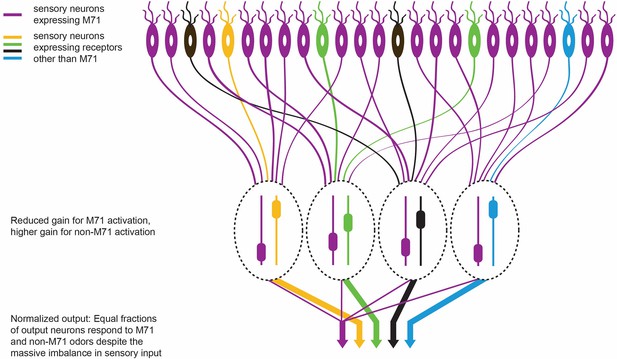
The olfactory response in M71 transgenic mice.
M71 transgenic mice are genetically modified to over-express the M71 receptor (shown in purple) in olfactory receptor neurons (top). However, mice can still detect other ‘minority’ odors because the neurons in the olfactory bulb (which receive signals from the olfactory receptor neurons) become less sensitive to signals from the dominant M71 receptors, and more sensitive to signals from other receptors (shown here in yellow, green, black and blue). The heights of the sliders in the ovals represent the level of gain in that circuit: high gain leads to high sensitivity. This ultimately enables each odor to produce an equal-sized output from the olfactory bulb (represented by the width of the arrows at the bottom of the figure).
One of the more unexpected findings of the 2008 study was the apparent inability of these mice to detect acetophenone: this was surprising because acetophenone (which is found in mouse urine) is the primary molecule that activates the M71 receptor. The study involved a behavioral task in which the animals had to discriminate unscented air from air that contained various concentrations of odors. The M71 transgenic mice were capable of quite subtle discriminations for all the tested odors except acetophenone.
Roland et al. now confirm the above behavior but also show that M71 transgenic mice start sniffing when exposed to acetophenone, suggesting that they can in fact detect this odor. If the earlier results were unexpected, the new data are even more intriguing and open up possibilities for teasing apart the mechanisms that underlie these apparently contradictory behaviors.
As a starting point, Roland et al. used a wide range of measures to examine what goes on at the output of the olfactory bulb in the M71 transgenic mice. For example, they used multiphoton imaging to record the activity of populations of neurons called mitral cells that transmit information from the bulb to the other olfactory regions of the brain. They also used whole-cell patch-clamp recordings to acquire detailed readouts from individual mitral cells in both anesthetized and awake mice. These measurements showed that the responses of the mitral cells in M71 transgenic mice are very similar to those of normal mice. The fraction of cells that respond to odors, including acetophenone, was indistinguishable from that seen in normal mice. The same was true for the time courses of the responses, and also for the dependence of odor-driven mitral cell activity on the mouse’s breathing patterns. The only apparent difference was that the output responses were more variable in the transgenic mice, and were slightly weaker to non-acetophenone odors.
This is rather impressive from a computational perspective. Not only does the olfactory system amplify responses to weak odorant inputs (that is, odors other than acetophenone) in the M71 transgenic mice, it also suppresses the massive sensory input (from acetophenone) that drives 95% of the sensory neurons (Figure 1). This normalization preserves much of the information about the detected odors despite the sensory input being hugely distorted.
How does the olfactory bulb accomplish this feat? Roland et al. trace the mechanism to a change in the balance of the inhibitory inputs to the mitral cells. These inputs increase in response to acetophenone, while there is less inhibition of other ‘minority’ odors than there is in normal mice. Based on the results of optical imaging, Roland et al. ascribe this change in the pattern of inhibition to cells called periglomerular cells that are found in the input layer of the olfactory bulb, but other explanations are also possible (Mori et al., 1999; Boyd et al., 2012; Kato et al., 2013; Miyamichi et al., 2013; Banerjee et al., 2015). Future work can build on the results of Roland et al. and isolate the contributions of the various olfactory circuit elements using modern genetic approaches and the large battery of tools that neuroscientists can use to manipulate neuronal activity.
One peculiarity that remains to be resolved is the discrepancy between the neuronal and behavioral responses of M71 transgenic mice to acetophenone. However, the study by Roland et al. sets up a powerful system for understanding the computations that occur in the first stages of the olfactory system to allow different smells to be detected.
In the language of electronic circuit theory, the olfactory bulb employs a “gain-control” layer early in processing to suppress large swings in the size of the input signals it receives. The outcome of such filtering (as seen in M71 transgenic mice) is exactly what one sees in an electronic circuit forced to find a small signal on a huge background. The circuit and bulb both become a little less sensitive and a little noisier. However, a typical electronic circuit has to do this for just one signal. The remarkable accomplishment of the olfactory bulb, as highlighted by Roland, Jordan, Sosulski, Fleischman and colleagues, is to do this for a variety of different signals.
References
Article and author information
Author details
Publication history
- Version of Record published: June 23, 2016 (version 1)
Copyright
© 2016, Gupta et al.
This article is distributed under the terms of the Creative Commons Attribution License, which permits unrestricted use and redistribution provided that the original author and source are credited.
Metrics
-
- 1,049
- views
-
- 132
- downloads
-
- 0
- citations
Views, downloads and citations are aggregated across all versions of this paper published by eLife.
Download links
Downloads (link to download the article as PDF)
Open citations (links to open the citations from this article in various online reference manager services)
Cite this article (links to download the citations from this article in formats compatible with various reference manager tools)
Further reading
-
- Neuroscience
Nociceptive sensory neurons convey pain-related signals to the CNS using action potentials. Loss-of-function mutations in the voltage-gated sodium channel NaV1.7 cause insensitivity to pain (presumably by reducing nociceptor excitability) but clinical trials seeking to treat pain by inhibiting NaV1.7 pharmacologically have struggled. This may reflect the variable contribution of NaV1.7 to nociceptor excitability. Contrary to claims that NaV1.7 is necessary for nociceptors to initiate action potentials, we show that nociceptors can achieve similar excitability using different combinations of NaV1.3, NaV1.7, and NaV1.8. Selectively blocking one of those NaV subtypes reduces nociceptor excitability only if the other subtypes are weakly expressed. For example, excitability relies on NaV1.8 in acutely dissociated nociceptors but responsibility shifts to NaV1.7 and NaV1.3 by the fourth day in culture. A similar shift in NaV dependence occurs in vivo after inflammation, impacting ability of the NaV1.7-selective inhibitor PF-05089771 to reduce pain in behavioral tests. Flexible use of different NaV subtypes exemplifies degeneracy – achieving similar function using different components – and compromises reliable modulation of nociceptor excitability by subtype-selective inhibitors. Identifying the dominant NaV subtype to predict drug efficacy is not trivial. Degeneracy at the cellular level must be considered when choosing drug targets at the molecular level.
-
- Neuroscience
Despite substantial progress in mapping the trajectory of network plasticity resulting from focal ischemic stroke, the extent and nature of changes in neuronal excitability and activity within the peri-infarct cortex of mice remains poorly defined. Most of the available data have been acquired from anesthetized animals, acute tissue slices, or infer changes in excitability from immunoassays on extracted tissue, and thus may not reflect cortical activity dynamics in the intact cortex of an awake animal. Here, in vivo two-photon calcium imaging in awake, behaving mice was used to longitudinally track cortical activity, network functional connectivity, and neural assembly architecture for 2 months following photothrombotic stroke targeting the forelimb somatosensory cortex. Sensorimotor recovery was tracked over the weeks following stroke, allowing us to relate network changes to behavior. Our data revealed spatially restricted but long-lasting alterations in somatosensory neural network function and connectivity. Specifically, we demonstrate significant and long-lasting disruptions in neural assembly architecture concurrent with a deficit in functional connectivity between individual neurons. Reductions in neuronal spiking in peri-infarct cortex were transient but predictive of impairment in skilled locomotion measured in the tapered beam task. Notably, altered neural networks were highly localized, with assembly architecture and neural connectivity relatively unaltered a short distance from the peri-infarct cortex, even in regions within ‘remapped’ forelimb functional representations identified using mesoscale imaging with anaesthetized preparations 8 weeks after stroke. Thus, using longitudinal two-photon microscopy in awake animals, these data show a complex spatiotemporal relationship between peri-infarct neuronal network function and behavioral recovery. Moreover, the data highlight an apparent disconnect between dramatic functional remapping identified using strong sensory stimulation in anaesthetized mice compared to more subtle and spatially restricted changes in individual neuron and local network function in awake mice during stroke recovery.